Talking Engineering: Dr Sanjay Vijendran
For nearly 7000 hours per year, Europe’s hundreds of millions of solar panels face a sunless sky. In the race to replace fossil fuels with sustainable alternatives, the European Space Agency (ESA) is heading a bold approach to provide permanent power by putting solar panels in space. Max Hersov spok
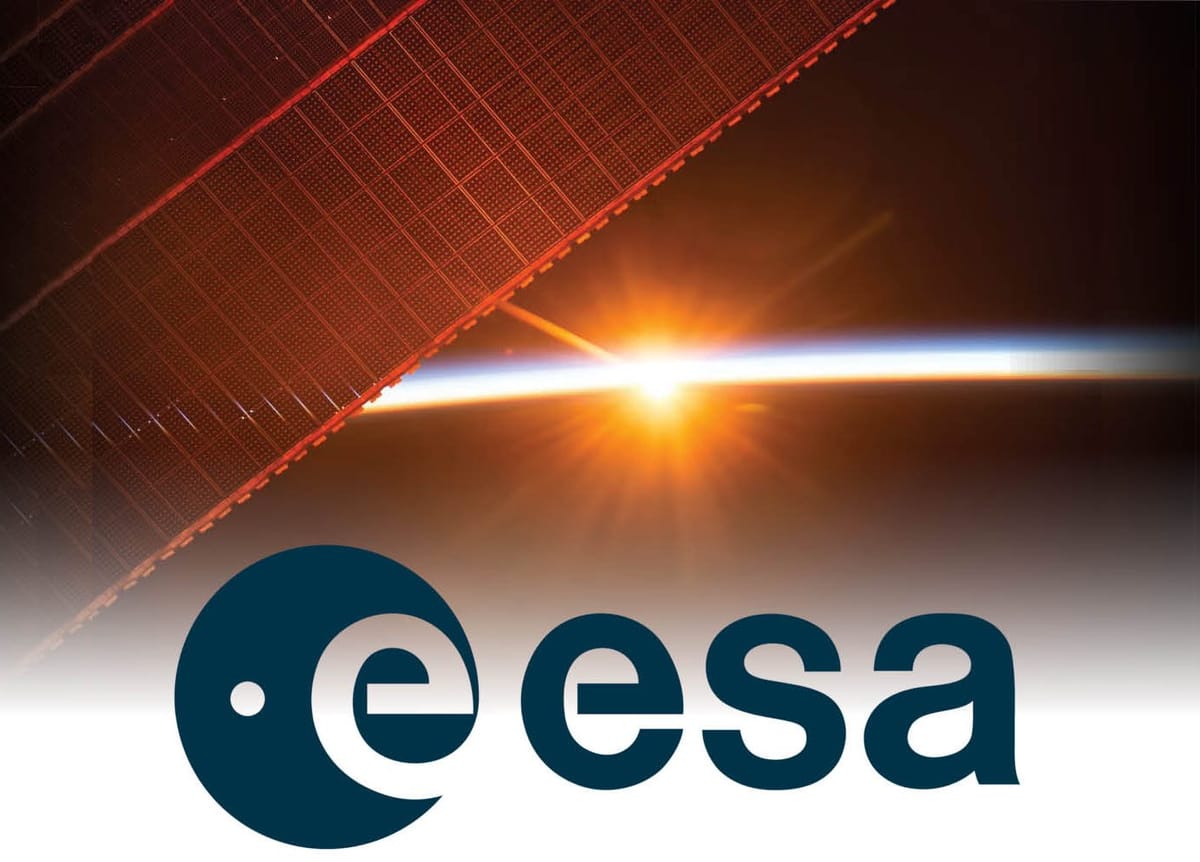
Legacy articles aren't reviewed and may be incorrectly formatted.
Talking Engineering 12: Dr Sanjay Vijendran Space-Based Solar Power
Conducted by Max Hersov, May 2023
For nearly 7000 hours per year1, Europe’s hundreds of millions of solar panels face a sunless sky. In the race to replace fossil fuels with sustainable alternatives, the European Space Agency (ESA) is heading a bold approach to provide permanent power by putting solar panels in space.
Max Hersov spoke to the Lead for the SOLARIS initiative on Space-based Solar Power and Mars Exploration Strategy Team Leader at ESA, Dr Sanjay Vijendran, who described ESA’s plan for a revolutionary new source of clean, affordable, continuous, abundant and secure energy to help accelerate the mitigation of the climate crisis.
Previously, Dr. Vijendran has served for more than a decade in ESA’s Mars Robotic Exploration Programme, planning and implementing the studies and technology development activities that prepare for future European robotic missions to Mars. He was instrumental in establishing in 2019 the international partnership between ESA and NASA on the multi-mission Mars Sample Return programme which is aiming to robotically return samples from Mars to Earth for the first time in the next decade. He also had the privilege of working as a payload development scientist on the NASA Phoenix Mars mission which landed on Mars in 2008. He holds a PhD in Semiconductor Physics from the University of Cambridge and has published widely on semiconductor devices and Mars exploration missions and technology.
MH: What is Solaris?
SV: Solaris is a new Research and Development (R&D) Initiative that we started at the European Space Agency (ESA) at the end of last year. It’s designed to be a three-year effort to mature the credibility of Space-Based Solar Power (SBSP) with some really detailed studies as well as technology development, so that we can get some hard data on the table about the feasibility. This will allow us to make a decision in 2025 on whether to go forward with a full development program for SBSP. So it’s a kind of preparatory step so that we can make a well-informed decision in the future.
There have been some decades of work by agencies all around the world including ESA on SBSP that show it’s very promising and likely to be technically feasible. However, it hasn’t been informed by significant hardware demonstrations so a lot of the analysis involves making assumptions on the performance of the technologies that we expect to improve with time. Now we really need to show that this is really possible by starting to develop these technologies rather than just assume that these are coming. So that’s why we are taking this step to invest more in the technologies for a period and then once we prove that there is a path to making this feasible, we can then take the decision to go ahead with a much larger investment.
MH: Is the plan to have solar concentrators which reflect/concentrate the sun’s light onto an area on the Earth’s surface for conversion into electricity there, or would you have the solar panels generate electricity in space and then beam it down via lasers or radio frequencies?
SV: The answer is that we are exploring both.
We have just started some system studies to look at the concept of a commercial-scale SBSP plants. One of the studies is going to be looking at a radio frequency - based power transmission method. There, you would have the solar panels in space, converting sunlight into electricity, which is then converted to radio frequencies, sent down using a huge antenna so that it’s in a reasonably small spot but still relatively large – some kilometers across on the surface of the Earth – where you have to collect it with a large receiver (known as a rectenna) that can convert it back into electricity. So, that’s the typical approach that many of the studies of the last couple of decades have tended towards, because it allows 24/7 power that is independent of the weather. This is because these frequencies that we use are in the Giga Hertz (GHz) range (similar to WiFi and cell phone frequencies), and these pass through clouds and rain without significant absorption. This means you can really have a 24/7 continuous source, day and night, through all seasons, to send that power down in a constant stream. This makes it much more reliable than terrestrial solar or wind, which is of course weather dependent.
Our other study will be looking at what is actually a simpler approach, which is to have very large mirrors in space – lightweight and thin – that can simply reflect the sunlight down onto the ground where you have your pre-existing solar farm, which is capturing sunlight when the sun is shining. You can add sunlight from these space mirrors during the day but more importantly you are able to provide sun at night to these solar farms when they are otherwise sitting unused. That way you can generate additional electricity on the ground and that makes it a lot simpler in terms of what you need to get into space. But, it does mean that you become weather dependent again, because you are just reflecting the sunlight, and for a location like Europe in the winter, that wouldn’t provide that much power, except on clear days. So this is why most focus is on the other method. However, in equatorial latitudes or areas where we know there are fewer clouds, then we can get much more power than relying on the normal day-night cycle and reduce the level of large-scale storage that is needed.
The main advantage of this reflection method is that it should be a lot simpler to launch and assemble the structure, because it can be a relatively light-weight structure and it doesn’t involve all the complicated steps of having the solar cells to convert into electricity, then converting that into radio-frequency, and having a huge antenna and beaming that antenna down in a proper accurate beam and then converting that back into electricity. All of this is very tricky to do. Simply having a mirror and pointing that in the right direction is expected to be a lot easier. But it’s disadvantage is that it doesn’t offer the same performance, the same 24/7 weather-independent application that the former microwave method does. So it has some niche applications in certain areas of the world, but is not suited for everywhere like the microwave approach.
MH: Besides the weather, what other advantages are there to the space-based microwave approach over terrestrial solar power generation?
SV: It’s the increased availability and intensity of the sunlight by going into space that makes all the difference. You get a lot more energy throughout a year, from a receiver on the ground which is receiving these microwaves 24/7 versus a conventional terrestrial solar farm.
This is because in terms of energy density, for every square meter of the land that you are using, the amount of energy you get is 2-3 times more than with conventional terrestrial solar power. Once you average solar power at northern latitudes across the day/night cycle, variable cloud cover and across all the seasons, you are effectively getting an average equivalent to something like 10 Watts per square meter (Wm-²) of continuous power into the grid. By comparison with the SBSP system, you can get 20-30 or more Wm-². Even this is not the maximum that you could get; it’s deliberately reduced to comply with what are known to safe limits of energy density at those frequencies. We ensure by design that the power at the edge of the receiver (also known as the rectifying antenna or rectenna) drops to a level that is safe for human exposure. We have calculated for a particular size of receiver something like 250 Wm-² in the centre would give you 10 Wm-² at the edge. Then you can be unrestricted in terms of human exposure. Where it is above 10 Wm-², you would have to have a fence around the receiver area and have restricted entry so nobody goes in and is exposed for a long period to these frequencies. So, the Physics would allow you to have more intensity and therefore get more energy for the land use, but there are other limits that we have to take into account and make sure that we have a safe and reliable system.
So, that’s one of the major benefits. You can basically get more energy per unit of land or use less land in the countryside to get the same amount of energy vs conventional terrestrial solar power. And secondly, very importantly, it’s a continuous form of electricity, which is in itself extremely valuable to the grid, because the grid wants to operate in a largely continuous way, since in our industrialised societies, we use energy pretty much the same day and night with only a relatively small difference.
MH: Are there any other risks? For example, the beam interfering with aircraft or other satellites?
Potentially, yes, and that is something we need to look into and understand a lot better. What studies have done so far in the design aspect is to limit that intensity of the beam to the levels I mentioned – less than about 250 Wm-² - and this is believed to be compatible with aircraft, spacecraft and living things such as birds that might be in the way of the beam. But this has not been well researched and tested with actual aircraft and satellites to see to what level there may be interference in communications or other implications. We need to confirm the impacts over a long-term exposure and whether there is any mitigation that can be put into place to either protect these things by designing adaptation into them or whether we would have to switch off the beam when something passes through it, or whether we would have no-fly zones, for example, although that’s rather extreme and ideally should be avoided. This needs to be studied further, but at least on paper it is believed that the power levels are not so intense that we would expect immediate damage to anything like a plane or a satellite.
MH: Now thinking about the actual solar panels in space, what are the difficulties or the differences when compared to panels on the ground? I’m thinking in terms of orientation, temperature fluctuations or solar radiation?
SV: Solar panels in space need to be designed specially for space because we want them to have a long lifetime. This is because historically, we have not generally been able to replace parts. We launch something, it runs its life, then that’s the end of it. So, we try to extend that lifetime as much as possible, and when we put satellites with solar panels into space we typically want them to last something like 15 years. And the reason there is an issue with lifetime in space is because it is quite challenging to make technology work in space due to the temperature fluctuations, the radiation coming from the sun and cosmic rays and all sorts of stuff flying about. Even small particles can do major physical damage. With respect to the radiation issue, normal solar cells that we use on Earth are designed only to handle very limited radiation that gets through our atmosphere, which is an extremely small amount compared to what you get up in space. So they work well down here but if you put the same cells in space they would very quickly get damaged by radiation and would not generate electricity after a matter of months or even less. Thus we have to design solar panels for space in such a way that they are “radiation hard”, requiring the use of special materials.
We also try to maximise the efficiency of these solar cells as in space the area that you have available to transport your solar panels is quite limited. Historically, your satellites have had to be very compact because they have to go up in one piece on a launcher – which until recently has been very costly to launch.. Thus the cells need to be as efficient as possible to have enough power for your space mission with a limited area of solar panels. Between the radiation hardness and the compatibility with the space environment as well as the high performance that we are looking for, space solar cells are custom-designed and very, very expensive compared to the ones we use on the ground. They are a much better quality and they can handle all sorts of extreme environments, but they come at a huge cost, typically a couple of orders of magnitude or more than for say the solar cells on your roof. The ones we use on earth are cheap, which is great, but they are only typically 20% efficient. We can accept the reduced efficiency for massively reduced cost. In the space market, we are often less driven by the cost of things and more driven by the performance and durability – it must provide a lot of power and last a long time.
For space-based solar power, we are looking at such a huge area of solar panels, for example 10 km² for one of these satellites, which is like a VERY large solar farm that you might see in the countryside. At the same time, in order to be commercially-viable you want to be selling this electricity at an affordable price while still making a profit. So now the cost becomes a major driver. It’s not just about having the most efficient cells, it’s about having the best combination between efficiency and cost that will make your electricity affordable, i.e. the cost per kilowatt-hour once you have delivered your electricity down to the ground. We already know that the best solar cells that we have for space use now are not the right ones to be used for SBSP because they cost way too much. So part of our Solaris research is to see whether we can reduce the cost of space solar cells by at least a factor of ten to make it more affordable. On the other hand, we are going to look to see how we can take the cells that we use on Earth (already low cost) and see whether we can adapt them to work in space without making them too expensive. By attacking the problem from two sides we’ll see where we end up.
MH: What materials are you looking at for the solar panels? Are you looking at just conventional silicon or perovskites?
SV: Yes, we are looking at all of the above. We are really taking a blank-sheet approach to what might be the best. Pure silicon is not expected to be suitable because it is sensitive to radiation so whether perovskites on top of silicon or something else which is a new and being researched for terrestrial solar panels because of the low cost and simple materials that you can source – whether that works out for the space environment is still to be seen.
We will be looking at the traditional space cell materials which are based on Gallium Arsenide, to see to what extent we can we bring costs down by mass production and new methods of production. And there may be novel materials that have not yet been considered for space use. Another aspect of this is energy security and having access to the raw materials in Europe, because we don’t want to trade getting our fossil fuels (where we are highly dependent on other countries) for some solar cell material which isn’t found in Europe and which we then have to buy from countries on which we don’t want to be dependent. That’s also an important factor that maybe we would not have thought about 30 years ago, but with all the events of the last year and the concerns about energy independence and security, this becomes an important consideration and a trade-off on top of the cost issue, efficiency, light weight, space radiation hardness, etc. Now we have to think about whether we can get hold of this material easily from friendly countries or even within European borders. A lot to juggle as we don’t want to trade one problem for another.
MH: So you have got to get a massive number of panels up - you said 10 km2 of solar panels per satellite. How many rocket loads would you say that amounts to? And how would they be assembled in space?
SV: Yes, and we want to have a large fleet of satellites because one power station is not enough for supplying an appreciable fraction of Europe’s needs We are talking about tens of them, or a hundred or more in the future. You need to put a lot of mass up into space.
How many rockets you would need is a function of how much mass each rocket can carry in a single launch. So, you can think about small to medium launches, up to super heavy-lift launches like SpaceX’s Starship. We know we will have to put up a lot of mass and we will have to put it up quickly if we want to make sure we can build these stations rapidly enough in order to help in time to impact climate change. Therefore we are looking at a very high cadence of launch - launches that can happen regularly, repeatedly and often, as well as carrying as much mass as possible per launch. Imagine a Starship heavy-lift launch happening a few times a week.
If you had something that could carry in the order of a 100 tons or more of payload into orbit in a single launch, you would still need of the order of 50 to 150 launches to put up enough mass, as well as the fuel that is needed, because when you do the launches you are usually launching initially to a lower orbit than where you might want to build the station Then you have to move it from the lower orbit to the higher orbit, using some sort of in-space tug. You need to fuel these tugs, and together with the hardware that needs to go up there, you need to also send a lot of fuel to do all these logistics.
In the end, if you are talking tens of satellites then it will require thousands of launches over the next decades. And that comes with high costs and complex logistics. You need lots of different rockets even if you can reuse them. Moreover, there is the issue of the environmental impact of these launches as well: when you are doing a small number of launches, as we have been doing over the last few decades, people have not been too concerned, but now, as we start to launch hundreds of rockets per year, not even a thousand per year, then you would be right to wonder what the negative impact of this is, particularly since the whole aim of SBSP in the first place is to have a positive impact by putting clean energy sources into space to displace fossil-fuel ones on Earth. Fortunately, that is an analysis that has been done in the past at a high level and it has shown that in principle, if you are shutting down a fossil fuel power plant by putting one of these systems into space, then you are saving so much CO2 emissions from shutting down the plant that it compensates overwhelmingly for the CO2 you emit from the launches as well as during the manufacture of all the necessary hardware and fuel. So there IS a net positive effect from a CO2 perspecitive. We need to update our analysis with the latest technologies and designs and confirm that this is still true and looks at wider environmental impacts that just CO2 as well. Obviously there is only a point in doing all this if it is going to have a net benefit regarding the planet. This is clearly a very important factor when we decide how we launch these things into space. Even the choice of the propellant for the launchers that we use; some give you a greener footprint than others. So we are also looking at what kind of future launcher we need to develop: should it be a hydrogen and oxygen combination, or could it be a methane and oxygen solution like Starship? We obviously want to minimize our environmental impact as we build and launch these systems.
A single, large coal-fired power station puts out around 15 Mega Tons of CO2 a year. SBSP, even if you consider all the launches, would put out less than 10% of that. Therefore, assuming a similar 30-year lifetime of the space-based solar-powered plant, you will have more than paid back the cost of the CO2 emitted within a few years. The CO2 pay-back time is even less than the solar panels on the roof of your house. These are mostly made in China, using fossil fuel and coal-fired power plants powering the production, and this produces emissions which take you something like 5 years on your roof in Europe to pay back in avoided CO2.. This is also because for more than 2/3rds of the time the panels are sitting there doing nothing - on cloudy days and at night. In space, the panels will be operating for more than 90% of the time. That is what makes it such a green solution, because you are really utilizing your investment in the hardware almost to the max.
MH: How will the solar panels be assembled and maintained in space?
SV: We hope to be able to do this fully robotically or with very minimal human intervention. The reason is simply to reduce the cost. In the past, SBSP didn’t go anywhere because the cost of it was prohibitive. Thirty and forty years ago, they could only imagine having lots of astronauts in orbit to assemble these things by hand. But now we are seeing robotic capabilities to service satellites in orbit, assemble them, repair them. These technologies have been in development for other applications, so we can easily spin these capabilities into this application. Obviously it would be at a much lager scale, but the basic technology – the ability to fly a spacecraft autonomously towards something, grapple it, move it to somewhere else, etc. - are capabilities coming online now. We can see the path to a fully autonomous in-space construction capability in the next ten years. Of course we need to look more deeply at applying it at the scale required, which nobody has really looked at before. But in terms of fundamental tech, we don’t think this is much beyond what is already state of the art.
MH: You mentioned already that small particles could be a risk. How will space debris be a factor in positioning the panels? Is that a risk at geostationary orbits?
SV: Yes, although that is less of a risk at geostationary compared to lower orbits, because there are fewer satellites there and they all tend to go around in the same direction, so you don’t tend to get this multitude of bits flying in at every direction, as happens in the lower orbits. Nonetheless, there is some risk and there are always micro meteorites that come from other parts of the solar system that pass through, and we do have to be conscious that with these large structures in space, the probability of being hit by stuff increases dramatically compared to normal satellites.
On the other hand, while we don’t want to suffer damage from the debris flying around and for it to affect our capacity to produce electricity, we also don’t want to be a source of new debris that rapidly escalates and causes a huge new problem. Both of these aspects are an important part of our study, to show we can build robustly and be compliant with our Zero Debris policy. ESA now has a Zero Debris policy where we want to make sure that from 2030 none of our missions will leave any debris in orbit at the end of life.
We have to make sure that we are not adding to the debris, and at the end of the 30 years, when we decommission the power station, we have to plan not to just leave it in space. A 5000 Ton satellite, that is 2km long, just left there or burnt up in the atmosphere would also be a tremendous waste of valuable materials. We have to think of a circular economy in which we re-use and recycle. We will have to design satellites so that they can be recycled in space into new SBSP stations. Otherwise this method of the power generation would not be sustainable.
MH: That would be a real break-through! It would also make it easier to get the raw materials in space if you’re already got most of the infrastructure there. Do you think this could become a reality on other planets, or on the moon? Do you think it could have uses there?
SV: SBSP may well be demonstrated in a real application on the moon first rather than on Earth because the scale that something like this is useful at is much smaller for exploration activities on the Moon or Mars than it is on Earth. Why is that? Because the Moon today has almost nothing going on and in the next twenty years it will be happy to receive anything from as little as 10 Kilowatts to at most 1 Megawatt of power. That will be a lot of power for a small base with limited activity. There won’t be a million people in a big city on the Moon in the next twenty years, which would require 100 Megawatts or even a Gigawatt of power. But on Earth that is typically your user base, unless you are looking at disaster zones or for the military where much smaller scales are really useful.
But if we are talking about climate change and providing power to several millions of homes, we need Gigawatts of power per solar power station. So that directly results in many thousands of tons of mass and ten square kilometers of area per satellite, but if you are looking at 10 Kilowatts or even a 100 kilowatts on the Moon, we can be that much smaller. It could be that a demonstration happens at the Moon in the next ten years, either by ESA or others, for a useful purpose on the Moon to power useful activities while also showing what is possible - that we get the infrastructure into space, we can assemble it, we can beam power, receive efficiently and we can scale up. Because it’s a small scale, it’s a proof of concept, and we then use that as a basis to scale up.
Similarly, on Mars, it also has uses. Mars like the Earth has an issue with dust storms and the day-night cycle, so if you had a base on Mars, it would be useful to have a reliable source of electricity that is not only nuclear power. This is an alternative.
A third application - in lower Earth orbit, which people are working on already - is beaming from satellite to satellite. Think of recharging satellites wirelessly by beaming from one satellite to the next. For instance, a larger satellite in high orbit in sunlight could beam to a satellite in low orbit which is in eclipse (in shadow of the Earth). In these periods they usually have to rely on batteries – and they have to size their batteries according to how long they are in that eclipse, and that is a big factor in determining the size and mass and cost of the satellite. If they could just receive power from a power station that sends power in the darkness, they would constantly have power and not need such big, heavy batteries.
The generic principle of being able to transmit power wirelessly is very useful for many, many applications, in space as well as on earth. There are a lot of benefits to not needing cables on Earth to send power.
MH: How close are we to a large-scale roll out?
This really depends on whether we have the political will and funding to make this happen quickly. At the current rate, the idea is receiving very small amounts of investment around the world, including the efforts of ESA. It is not major. It is more than has happened in the past but it’s not really commensurate to what other major investments are going into other clean energy technologies. So, if we leave it at this current rate, it can easily be 20-30 years, or even more. BUT, if we really put effort into it and the right amount of resources, about $15-20 billion, to do the R&D and develop the first full-scale station, then we think this could be done in 10-15 years after a decision is made to go ahead. An all-hands-on-deck approach is required if we really want to do this in the timeframe that really matters for the climate crisis. The physics is well understood. It’s not a scientific problem, it’s more an engineering problem. Because of the scale of it. Every telecom satellite is effectively a solar power beaming satellite. Step by step, the physics is exactly the same. It’s just that that is sending small amounts of power. We need to send a much more directed beam at many orders of magnitude larger scale. We just need people to work on it hard. It has not received that level of visibility yet as it’s been for too long in the realms of science fiction and only known to the space sectro. It’s really the energy sector people who have the problem of delivering Net Zero and clean energy – they need to wake up and see that this is a real potential energy source. If they do, they have plenty of money to pour into something like this and get it done. We in the space industry now need to do our bit to show that there is a path to technical feasibility, but we are challenged by our sources of funding not being that great. It’s moving but too slowly.
MH: Thank you so much for your time. It’s been incredibly interesting. Do you have anything to add?
SV: I want to elaborate on a few things - the number of launches required for satellite solar power stations, recycling in space and the efficient use of resources.
We were saying that about 50 satellites might already result in having some 1000’s launches. If we are to develop and deploy SBSP further, so that it starts to provide a good fraction of the world’s energy we might really want to have 1,000 of these satellites - that is a LOT of launches! (By comparison we have 300 coal power stations and 100 nuclear power stations in Europe alone today and there are tens of thousands of power stations around the world). Even if we minimize the environmental impact, there is still going to be a significant impact from tens of thousands of launches per year, so can we find a way to minimize it even more? One way to do that would be to avoid having to lift all this material from the surface of the Earth, but rather to lift it from the surface of the Moon. What about using the Moon’s resources to build the hardware, or at least most of it, and then launching that from the Moon into Earth orbit for it to be assembled with any other bits required from the Earth? Launching things into Earth orbit from the Moon requires a lot less energy than launching it from the Earth itself. We are studying for the longer term, what materials there are on the Moon that we can utilise to make solar cells as well as structural parts and whether we can launch those from the Moon using rockets that are getting fuel from the Moon as well. This way we would limit the number of launches required from the Earth and that is possibly a way both to reduce the environmental impact on the Earth as well as the cost. This provides a longer-term perspective and another interesting reason for going to the Moon, to explore whether it possible to use the Moon for such projects. There really appears to be a good case to do this in the future. It’s not something for the next twenty years, but rather something for the balance of the century thereafter.
Many thanks to Dr Vijendran for his valuable time and insight.
1 https://www.currentresults.com/Weather/Europe/Cities/sunshine-annual-average.php