Quark-Gluon Plasma
To many, prime numbers are a useless concept studied in maths and soon forgotten about, having no impact on the rest of our lives. While they do have some applications in computing and encryption, primes never seem to crop up in day-to-day life, remaining as “special numbers” that aren’t all that sp
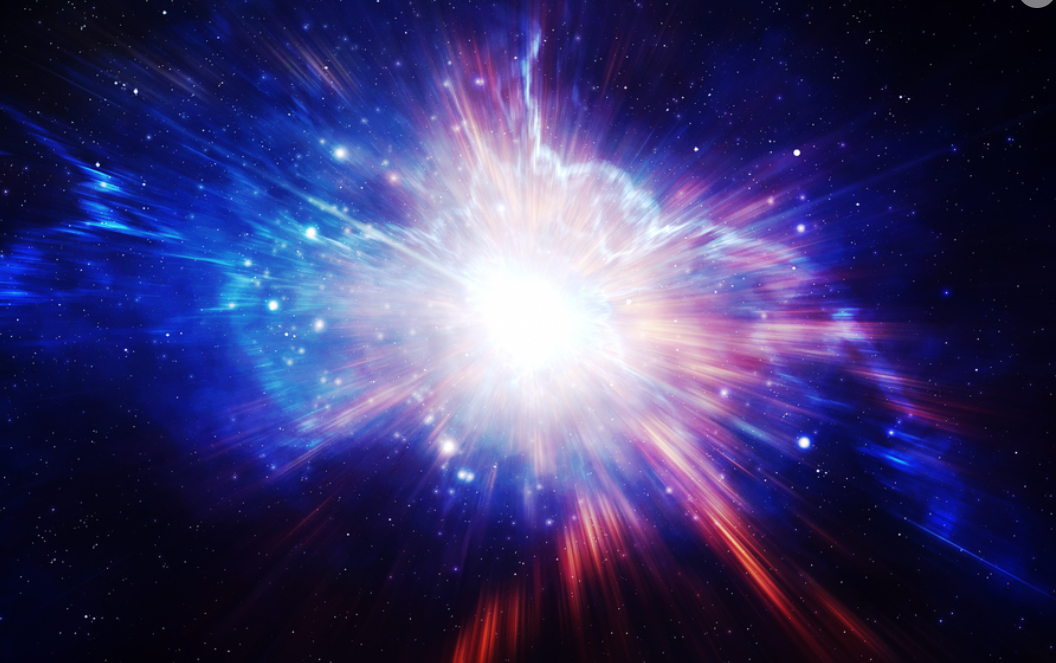
Legacy articles aren't reviewed and may be incorrectly formatted.
Quark-Gluon Plasma
Michael Wu
Quark-Gluon Plasma (QGP) is a unique state of matter that is believed to have existed in the early
universe microseconds after the Big Bang. Thus understanding the properties and formation
mechanisms of QGP provides us with crucial insights into the fundamental nature of matter and
subsequently, the evolution and growth of the universe. It is a localized assembly of quarks and
gluons at thermal and chemical equilibrium, alternatively described as a 'soup'. (Letessier & Rafelski,
2002) This project aims to explore the theoretical framework from which current information on
QGP is built upon, the experimental techniques which have been deployed in studying this
phenomenon and what prospects this holds for the future.
First, it is important to define and understand the components of QGP; quarks are a type of
elementary subatomic particle carrying a fractional electric charge and are postulated to be the
fermionic component of hadrons in classical quantum chromodynamics. Similarly, gluons are also
subatomic particles believed to transmit the binding force present between quarks to form hadrons,
known as the bosonic component. The hadrons which are formed from these elementary particles
combine to form protons and neutrons: terms which are more recognisable to be present in all
elements. Despite there being no direct observation of quarks and gluons, theoretical predictions
based upon their existence have been experimentally confirmed. Quarks were first conclusively
proven to exist in 1968 at the Stanford Linear Accelerator Center (Taylor, 2000) whilst gluons were
proven to exist in 1979 by John Ellis in CERN. (Ellis, Gaillard, & Ross, 1976)
Quark-Gluon Plasma is a particular state of matter involving these elementary particles where they
are freed of their strong forces of attraction between each other in the formation of hadrons, only
possible under extremely high densities. (Ericson, Jacob, Satz, & Willis, 1982) The exact value of the
density is around five to ten times the density of nuclear matter, corresponding to circa. 2.0 x 10^15
g cm^-3. The alternative condition would be to increase the temperature to about 10^12 Kelvin,
which is 70,000 times hotter than the centre of the Sun. Under normal conditions, quarks are bound
as hadrons, but in QGP, they can exist in free form. (Sementana, 2018) The binding of quarks is
called confinement, which under the abovementioned specific conditions, are broken up in a
transition known as deconfinement phase transition. With reference to quantum chromodynamics,
the quantum field of strong interactions, these quarks interact with each other via the exchange of
gluons, which continue to form a plasma-like conglomerate with the free form quarks- Quark-Gluon
Plasma. (Letessier & Rafelski, 2002)
Where is this particular state of matter relevant? The Big Bang Theory is commonly accepted to be
the model of the formation of the Universe. In the immediate timeframe after this occurrence, the
extreme conditions (high density and temperature) were met and quarks and gluons existed freely,
unconfined by protons and neutrons. This specific type of QGP is regarded as a ‘hot’ QGP since the
fireball of the Big Bang was small and hot in order to support the formation of this matter. Another
occurrence of this phenomenon is hypothesized to exist as a ‘cold’ QGP in compact objects such as
neutron starts or quark stars, but there is lack of research to back up any of these claims. By
understanding the exact nature of how our universe began, scientists aim to comprehend and hence
predict the evolution of the universe: an achievement which will change the course of the future of
human existence.
Many physicists have attempted to produce this quark-gluon plasma experimentally in particle
accelerators ever since this phenomenon first came to light four decades ago, essentially attempting
to simulate a big bang in the laboratory. Experiments to create this artificial deconfined quark
matter first started at CERN in 1986/87, and after 5 years the first claims and hypotheses were made
(eg production of multistrange baryons and antibaryons in sulfur-tungsten interactions at 200 GeV/c
per nucleon). (Abatzis, et al., 1990) Years later, experiment WA97 at CERN presented a new state of
matter in heavy ion collisions with a “perfect liquid” of quarks and gluons produced using the
collisions of gold nuclei. (Jacak & Steinberg, 2010) This was later elaborated by Brookhaven National
Laboratory’s Relativistic Heavy Ion Collider (RHIC). (Laboratory, 2024) Efforts in conducting key
experiments to continue exploring the properties of QGP are being continued at the RHIC and at
CERN’s Large Hadron Collider (LHC), by colliding relativistically accelerated gold and other ions
with each other or with protons. Another element being commonly used in these experiments are
lead ions which were used for the ALICE experiment in 2011 at CERN, where a record breaking
temperature was set in the ranges of 5.5 trillion kelvin. (2024) These heavy elements are suitable for
these experiments because they already bring many particles with them for a collision, have high
kinetic energies when accelerated (kinetic energy grows linearly with mass) and have a larger cross
section for collision. The heavy ions are shot at each other and are intended to generate the QGP in
a tiny point in space for extremely short periods of time: the energy density is the central parameter
in particle accelerator physics. The aim is to deposit a lot of energy (in the form of fast particles) in a
small volume.
Despite the hardships of replicating the extreme conditions necessary in particle accelerators, the
discovery and experimentation on quark-gluon plasma illustrates the journey physicists have
undertaken to reach a point where glimpses of the early universe and potentially the future are
within grasp. With technology continuously being developed, the study of QGP will act as a frontier
in the exploration of the very nature of our existence.
References
2024, January 26). Retrieved from https://web.archive.org/web/20160304203826/http://blogs.nature.com/news/2012/08/hot-stuff-cern-physicists-create-record-breaking-subatomic-soup.html
Abatzis, S., Antinori, F., Barnes, R., Benayoun, M., Beusch, W.,
Bloodworth, I., . . . Evans, D. (1990). Production of multistrange baryons and
antibaryons in sulphur-tungsten interactions at 200 GeV/c per nucleon. CERN.
Ellis, J., Gaillard, M. K., & Ross, G. G. (1976). Search for gluons in e+ e- annihilation.
Ericson, T. E., Jacob, M. R., Satz, H., & Willis, W. J. (1982).
Quark matter formation and heavy ion collision. CERN.
Jacak, B., & Steinberg, P. (2010). Creating the perfect liquid
in heavy-ion collisions. Physics Today,
39-43.
Laboratory, B. N. (2024, January 25). Relativistic
Heavy Ion Collider. Retrieved from https://www.bnl.gov/rhic/
Letessier, J., & Rafelski, J. (2002). Hadrons and Quark-Gluon
Plasma. Cambridge University Press.
0521385369_frontmatter.pdf
(cambridge.org)
Sementana, T. (2018).
Taylor, R. E. (2000). The Discovery of the Point-Like Structure of
Matter. Stanford, 1-18.