From Commensal to Pathogen: The Mechanisms Behind Bacteria
Explore the roles of host susceptibility, virulence factors, genetic adaptation, and environmental triggers in the sudden change of the bacteria from commensal to virulent
Abstract
The concept of commensal bacteria began with Antonie van Leeuwenhoek, who conducted pioneering observations in the late 17th century using the first microscopes. He was the first ever man to describe the presence of microscopic organisms in human oral and fecal samples and unknowingly documented the existence of commensal microbes (van Leeuwenhoek, 2000). For centuries, these microorganisms were thought to be just passive, coexisting on mucosal surfaces, the skin, and the gastrointestinal tract. However, as the field of microbiology advanced through the work of Louis Pasteur and Robert Koch in the 19th century, the relationship between humans and microbiota began to unfold (Falk et al., 1998). By the mid-20th century, researchers discovered that these bacteria have a vital role in maintaining homeostasis, contributing to immune system development, nutrient metabolism, and protection against invasive pathogens (Belkaid & Hand, 2014). Despite these beneficial roles, it has also been found that having these bacteria can have consequences, as under certain conditions, they can undergo a transition into opportunistic pathogens, exploiting host vulnerabilities and leading to infection (Nguyen et al., 2015).
This essay explores the role of host susceptibility, virulence factors, genetic adaptation, and environmental triggers in the sudden change of the bacteria from commensal to virulent.
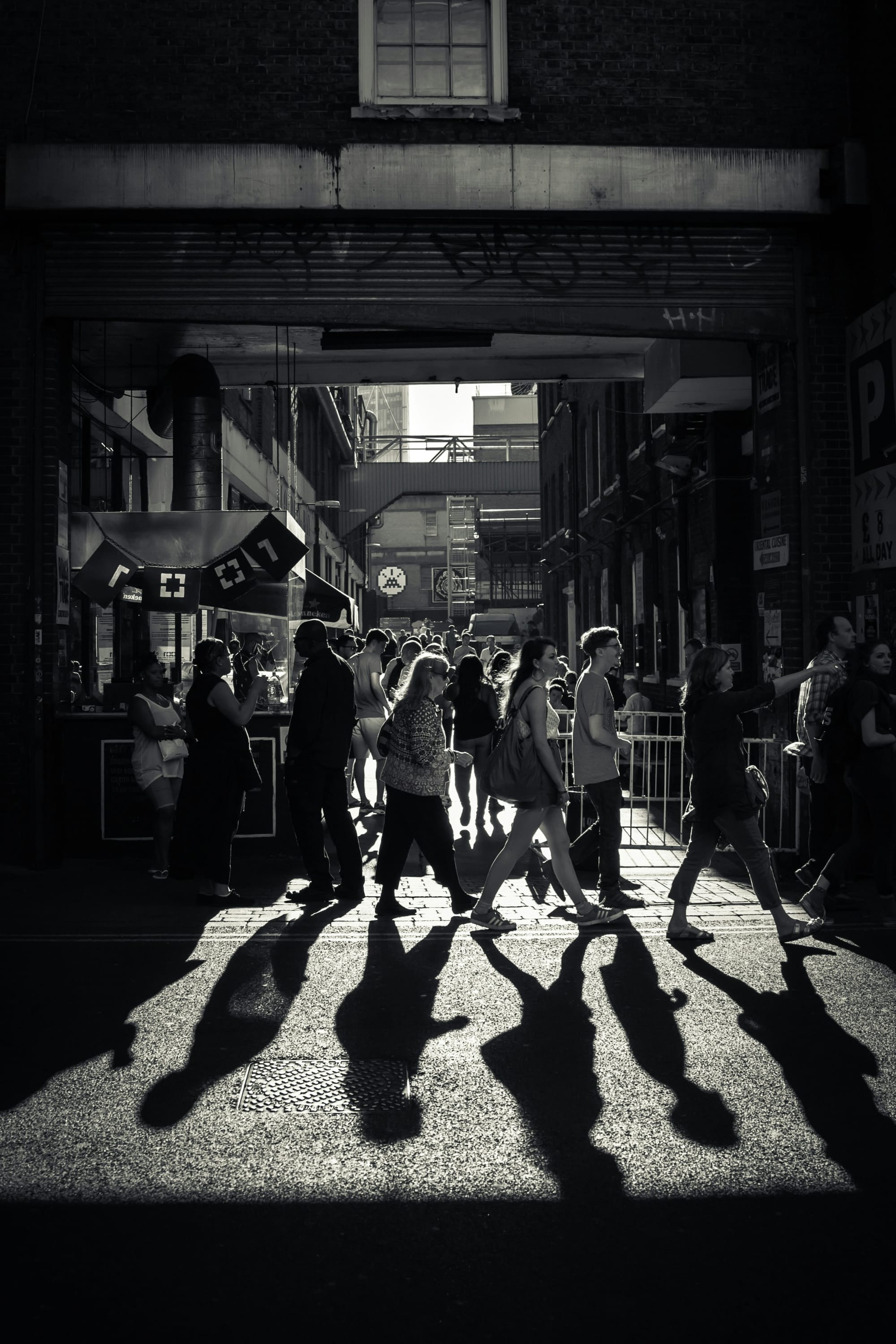
Human Compromise
Section One
The transition from commensal to pathogenic bacterium is often heavily determined by host susceptibility. In healthy individuals, the immune system employs physical barriers, phagocytic cells, and humoral immunity to prevent bacterial growth and invasion. (Mims et al., 2004). However, immunocompromised states such as those induced by chemotherapy, organ transplantation, or diseases like HIV/AIDS significantly increase susceptibility to bacterial infection (Finlay & Falkow, 1997).
For example, Escherichia coli (E. coli), a typical commensal organism of the gut, frequently causes urinary tract infections in patients who have undergone catheterization, which is when a tube is inserted into a body channel. Here, the loss of mechanical barriers and the weakening of innate immunity allow for infection. (Wilson et al., 2002). Similarly, burn victims are highly susceptible to Pseudomonas aeruginosa, which takes advantage of damaged epithelial defenses to infiltrate deeper tissues and have a greater growth rate (Pier et al., 2001).
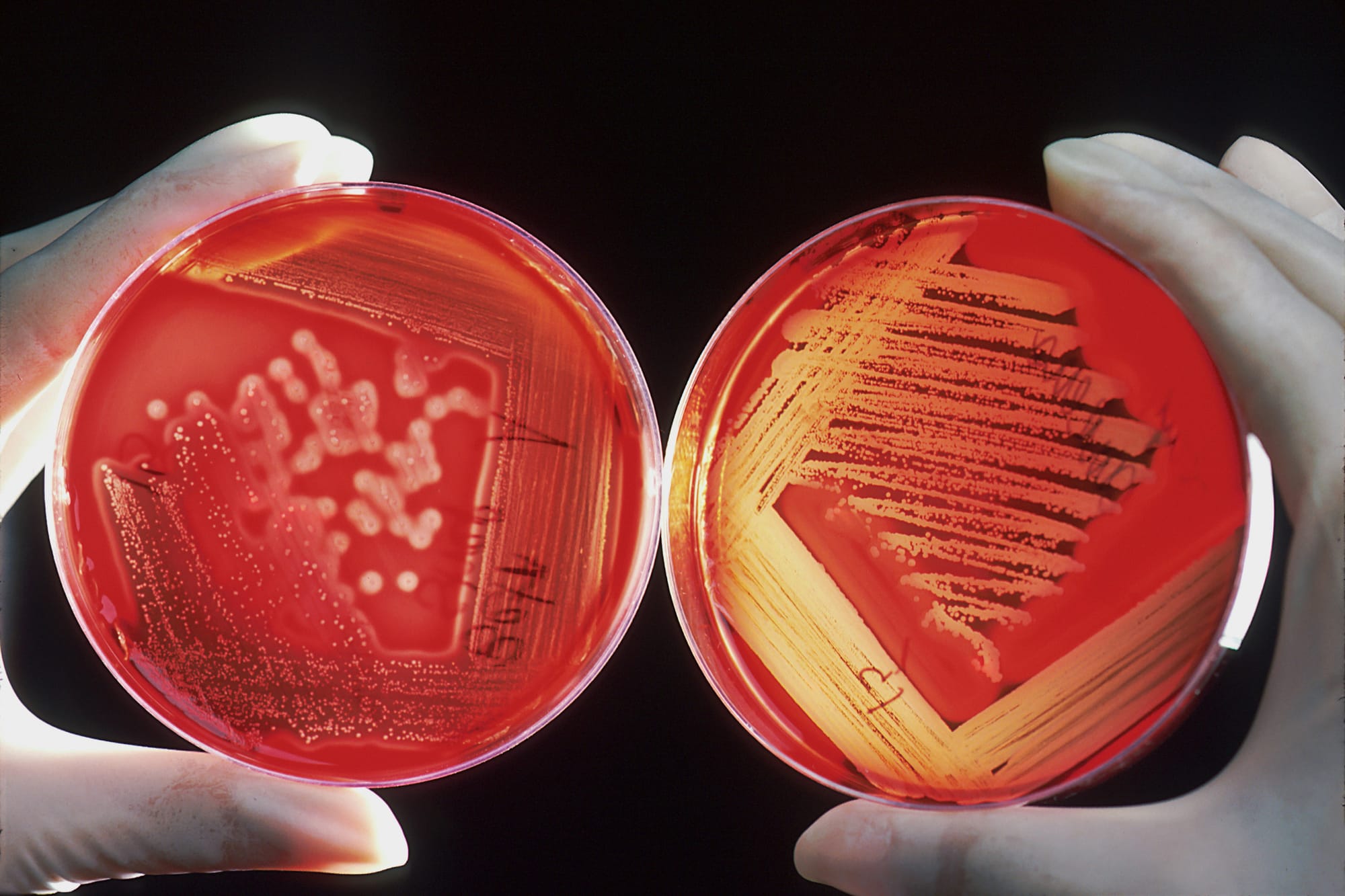
Molecular Tools
Section Two
A defining characteristic of pathogenic bacteria is the possession of “virulence factors”—molecular tools that enable invasion, evasion of immune responses, and tissue damage (Casadevall & Pirofski, 1999). These factors are typically acquired through horizontal gene transfer (HGT) mechanisms, which include using plasmids, bacteriophages, and transposons for either transformation, transduction, or conjugation, which can be seen in Figure 1 for Bartonella (Hacker & Kaper, 2000).
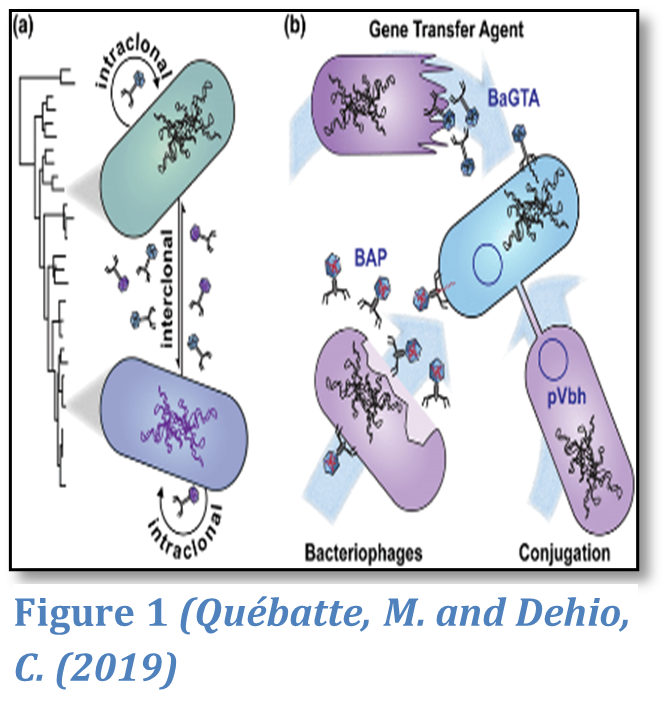
An example of this is Staphylococcus epidermidis, which is a skin commensal that can become pathogenic by gaining genes by any HGT mechanism, allowing them to form biofilms.
Biofilms shield the bacteria from the immune system and antibiotics, allowing for persistent infections, most commonly inside implanted medical devices such as pacemakers (Vuong et al., 2004).
Possibly the most essential tool for infection is toxins, which cause damage to us in the first place. Bacterial toxins are typically classified under two major categories: exotoxins or endotoxins. Exotoxins are immediately released into the surrounding environment, whereas endotoxins are not released until the bacteria are killed by the immune system.
An example of this is in some strains of E. coli, such as Enterohemorrhagic E. coli, which have evolved to produce Shiga toxins, a potent exotoxin that causes severe gastrointestinal disease. This occurs when E. Coli acquires a Shiga-toxin-encoding gene from bacteriophages through transduction. (Eslami et al., 2021). This toxin is an AB5 toxin, which is a six-component protein complex consisting of an A subunit that inhibits ribosomal activity, leading to host cell death and a B subunit that binds to receptors on the host’s endothelial cells, facilitating toxin entry. Upon entering the host, Shiga toxin blocks protein synthesis and destroys vascular endothelial cells, leading to hemorrhagic colitis. It can also trigger systemic complications, including hemolytic uremic syndrome, which causes kidney failure due to widespread endothelial damage.
This extremity of damage is interesting as E. coli strains lacking this phage-acquired toxigenic gene remain completely harmless and beneficial to gut activity.
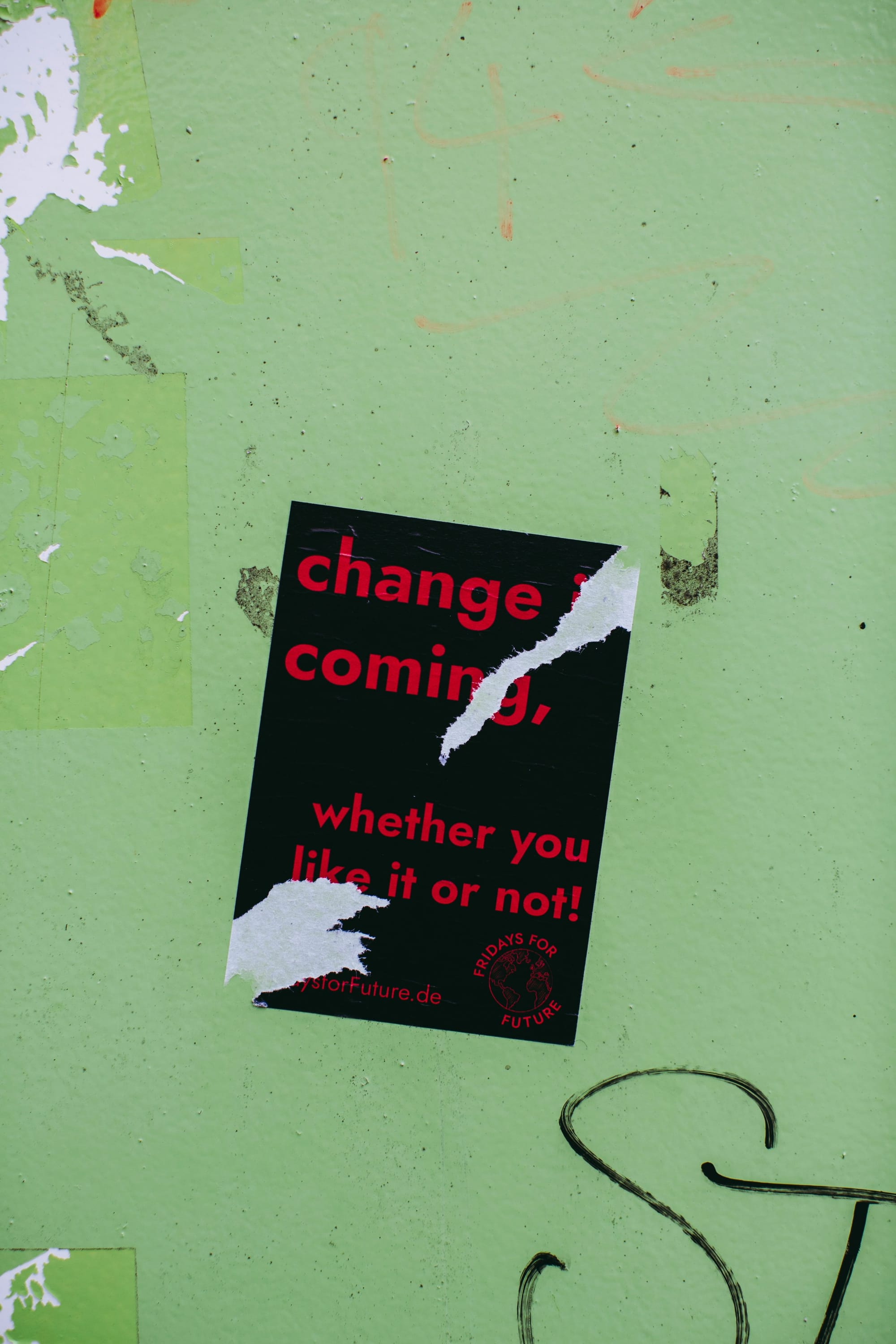
Inducing Change
Section Three
Environmental conditions play a key role in the adaptation of commensal bacteria into pathogenic organisms. Bacteria must continuously adapt to the dynamic environment within the host, where changes in temperature, pH, oxygen levels, and nutrient availability can trigger stress responses. These responses can activate genes responsible for virulence. (Goh et al., 2013).
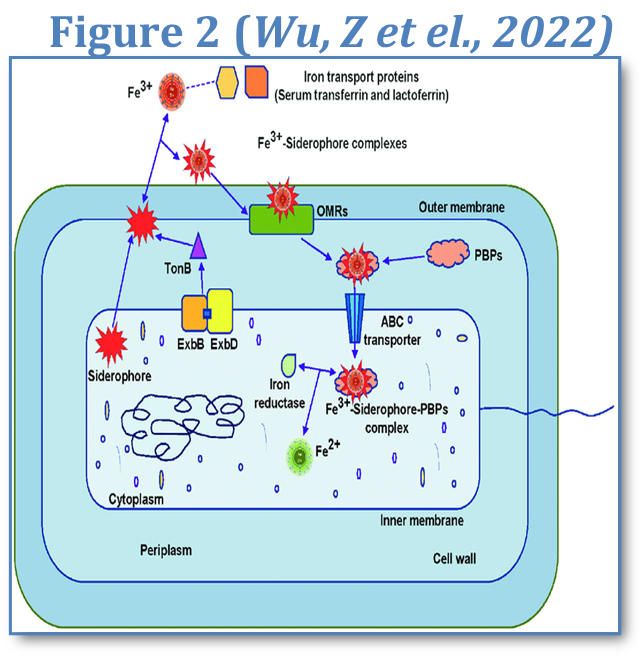
For instance, under iron-limited conditions-which are common within humans, many bacteria increase siderophore production, which allows them to increase iron intake for growth and therefore survival, which causes harm in humans due to a depleted iron concentration and increased cell death. (Ratledge & Dover, 2000). This mechanism can be seen in Figure 2.
Klebsiella pneumoniae, a gut commensal, uses siderophores to be able to invasively infect humans, particularly in immunocompromised patients (Paczosa & Mecsas, 2016; Holden et al., 2016).
This change in condition can also be achieved by other pathogens, such as how, in the respiratory tract, mucosal damage due to viral infections can create conditions perfect for bacterial superinfection, as seen with Streptococcus pneumoniae following influenza (Peltola & McCullers., 2004).
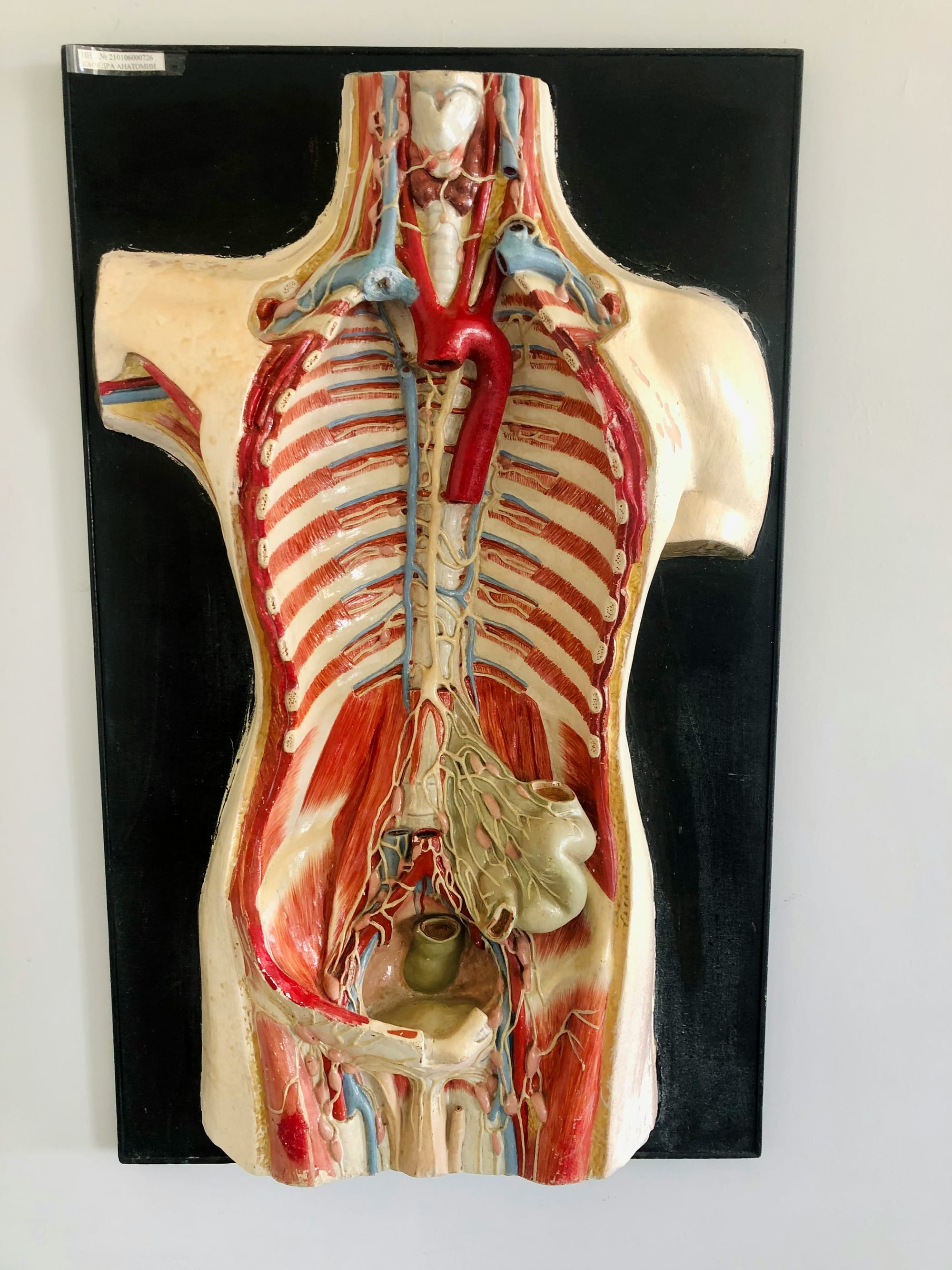
Becoming Pathogenic
Section Four
Commensal bacteria can become pathogenic by acquiring genetic elements that encode proteins facilitating cell invasion. Many bacteria use specialised structures, such as pili or adhesins, to bind to host receptors and trigger their uptake (Finlay & Falkow, 1997).This ability allows bacteria to cross epithelial barriers and access sterile body compartments. For example, E. coli can cause urinary tract infections by using type 1 pili to adhere to and invade bladder epithelial cells (Mulvey et al., 2000). The genetic basis for these adaptations is again via HGT mechanisms.
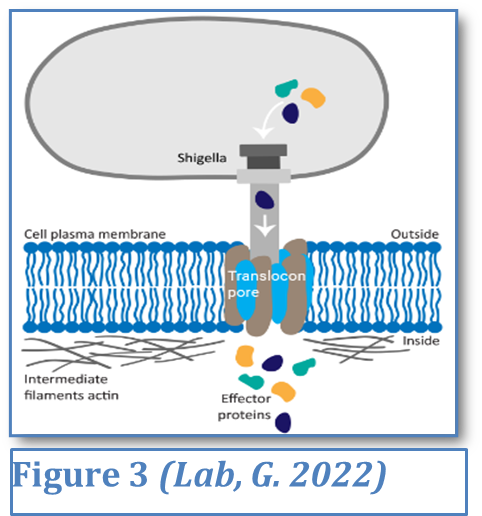
A key mechanism in bacterial virulence is the Type III secretion system, a needle-like structure used by pathogens such as Salmonella enterica to inject effector proteins into host cells. This can be seen in Figure 3. These proteins manipulate the host’s cytoskeleton, forcing the cell to engulf the bacterium and effectively allowing it to invade tissues. This strategy not only aids infection but also enables bacteria to evade immune detection by hiding inside host cells—an evolutionary advantage that contributes to their persistence in the body. Although most Salmonella strains are commensal in animals, these invasive variants cause severe human disease by breaching intestinal barriers (Galan & Curtiss, 1989).
Certain bacteria make use of intracellular niches- an environment where intracellular bacteria can survive and proliferate- to evade immune detection and antimicrobial agents. Pathogens like Salmonella enterica and Listeria monocytogenes have evolved mechanisms to survive within phagocytic cells, escaping lysosomal breakdown (Powers et al., 2021). For instance, Legionella pneumophila, a waterborne bacterium, is usually harmless in natural environments, but becomes pathogenic when inhaled into the human lungs. It survives by inhibiting phagosome-lysosome fusion, leading to Legionnaires’ disease (Horwitz, 1983). Such adaptations not only enhance bacterial survival but also contribute to chronic and recurrent infections.
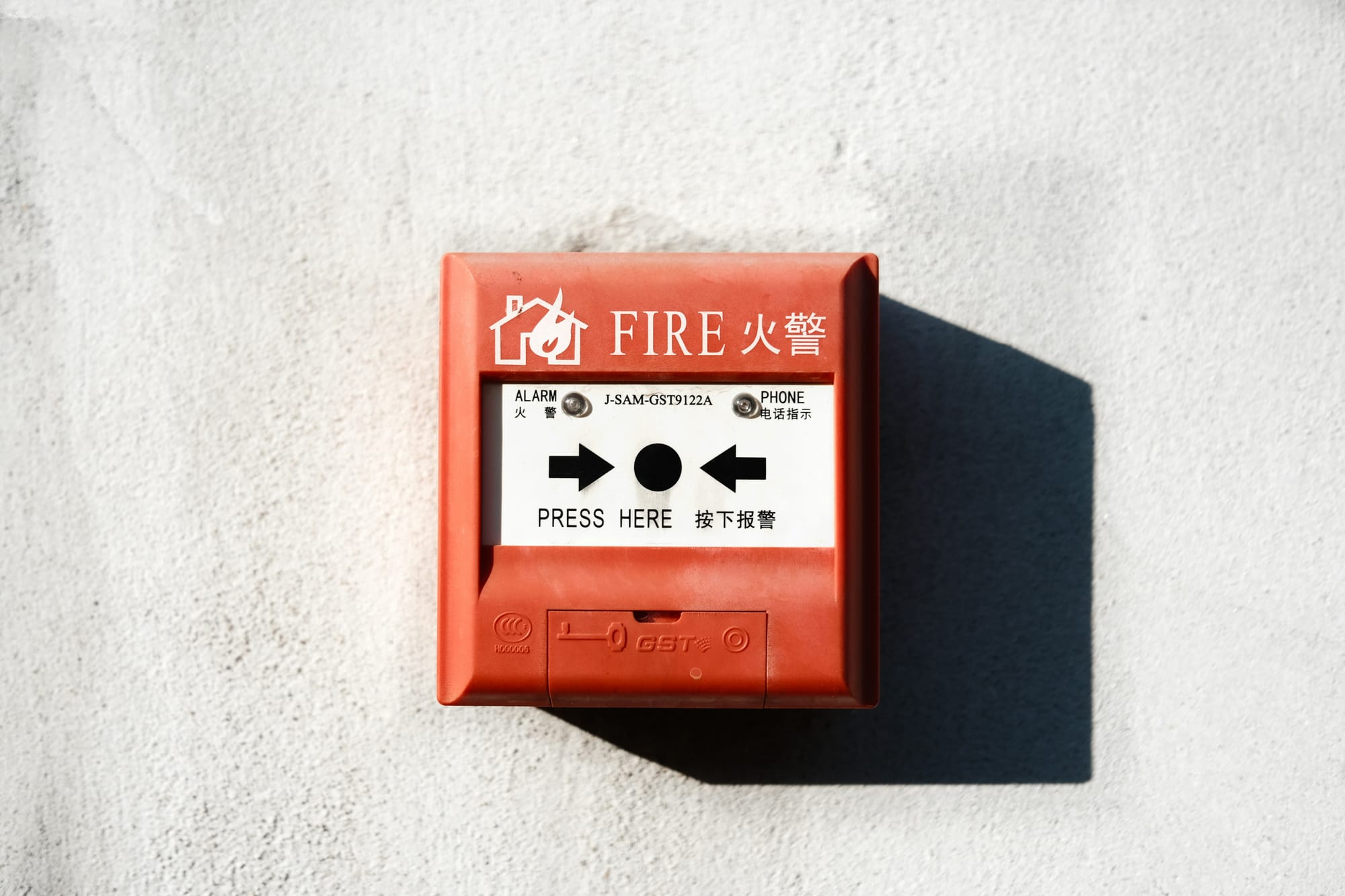
Friendly Fire
Section Five
Interestingly, the immune system itself can contribute to bacterial pathogenicity. Excessive immune responses, as seen in sepsis caused by gram-negative bacteria, lead to tissue damage through inflammatory cytokine release (Van Amersfoort et al., 2003). In diseases like tuberculosis, much of the pathology is due to the host’s response, rather than from direct bacterial toxins (Flynn & Chan, 2001).
This is applicable for commensals as certain members of the gut microbiota, such as E. coli, under conditions of immune dysregulation, can trigger excessive cytokine release, contributing to inflammatory diseases like ulcerative colitis (Plichta et al., 2019). Similarly, Bacteroides fragilis, a commensal anaerobe of the gut, can be a large cause of inflammatory bowel disease due to its ability to activate immune responses through its polysaccharide A antigen, a type of antigen that is usually found on the outside of things like cells or viruses (Mazmanian et al., 2008). Antibiotic pressure can force bacteria to develop resistance genes, resulting in a bacterium which cannot be treated if it becomes pathogenic. For example, the widespread use of broad-spectrum antibiotics disrupts normal microbiota, allowing opportunistic pathogens such as Clostridioides difficile, which disrupts the gut microbiota, causing severe colitis, an inflammatory condition of the colon characterised by persistent diarrhoea and mucosal damage (Theriot & Young, 2015).
Another example of this is Enterococcus faecium, once a harmless gut commensal, which has evolved into a leading cause of nosocomial infections, due to its vancomycin resistance (Arias & Murray, 2012). Vancomycin is a widely used antibiotic in the treatment of Staphylococcus aureus (MRSA).
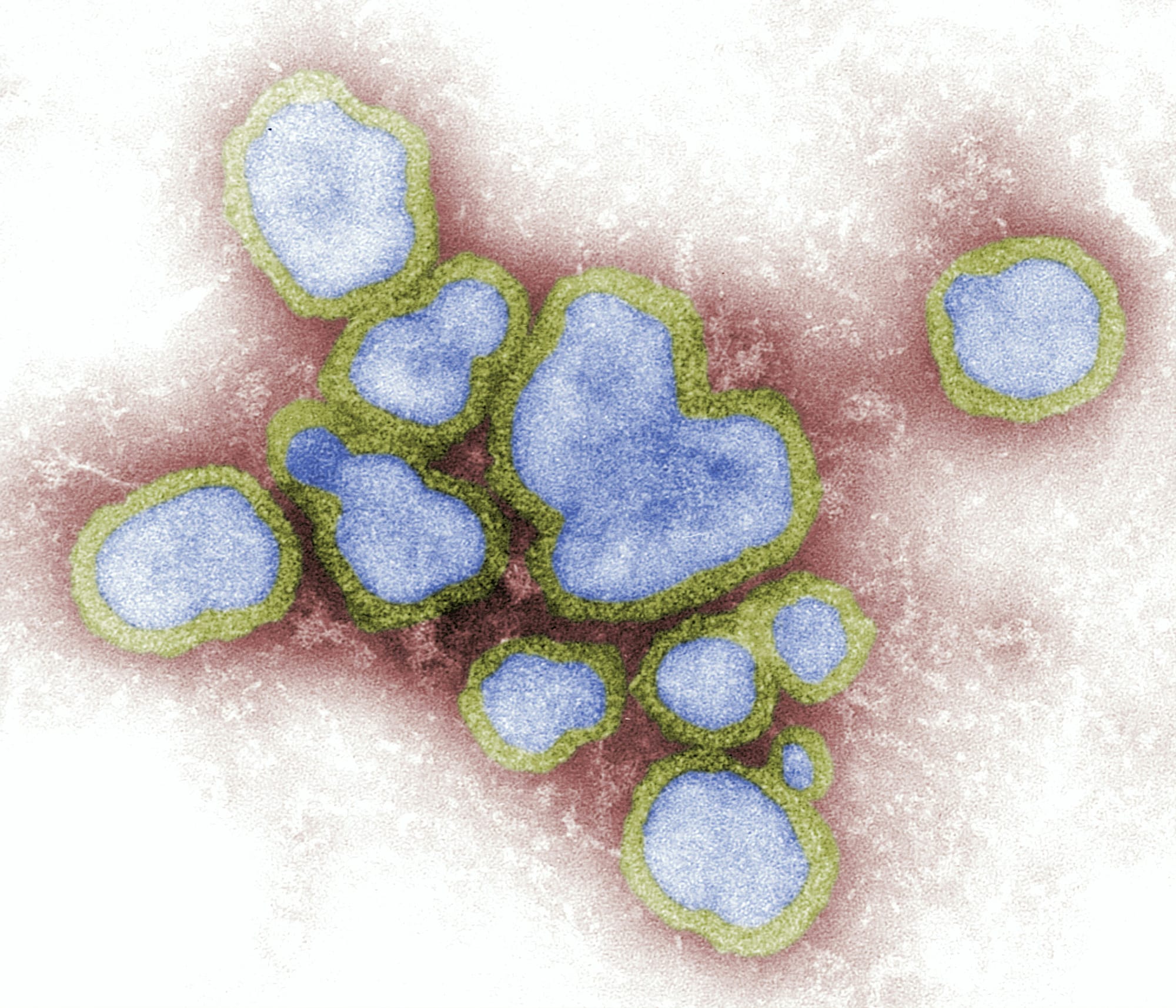
Mechanisms For Immunity
Section Six
An example of a mechanism by which commensal bacteria become resistant to antibiotics is enzymatic degradation. This is present in many gut and skin commensals, such as E. coli and Bacteroides species, where β-lactamases will be produced, which can break down β-lactam antibiotics like penicillin and cephalosporins (Bush & Bradford, 2016). While these bacteria are normally harmless, prolonged antibiotic exposure allows strains to develop with enhanced β-lactamase activity, increasing the likelihood that resistance genes will spread to opportunistic pathogens.
For example, Enterococcus faecium, a commensal in the gut, has developed resistance to multiple antibiotics such as vancomycin, which can cause severe bloodstream infections in hospitalised patients.
Another mechanism of resistance is Efflux pumps, which originally evolved to remove toxins and have now adapted to allow for resistance to fluoroquinolones, tetracyclines, and macrolides, which are broad-spectrum antibiotics.
Overuse of antibiotics in areas such as medicine and agriculture has increased efflux-mediated resistance, allowing commensals to survive and transfer resistance genes to harmful pathogens like Klebsiella pneumoniae or Salmonella (Martínez et al., 2014).
Interestingly, some gut bacteria, such as Bacteroides fragilis and E. coli, use multidrug efflux pumps to expel antibiotics before they reach lethal concentrations and kill the bacteria (Blair et al., 2015).
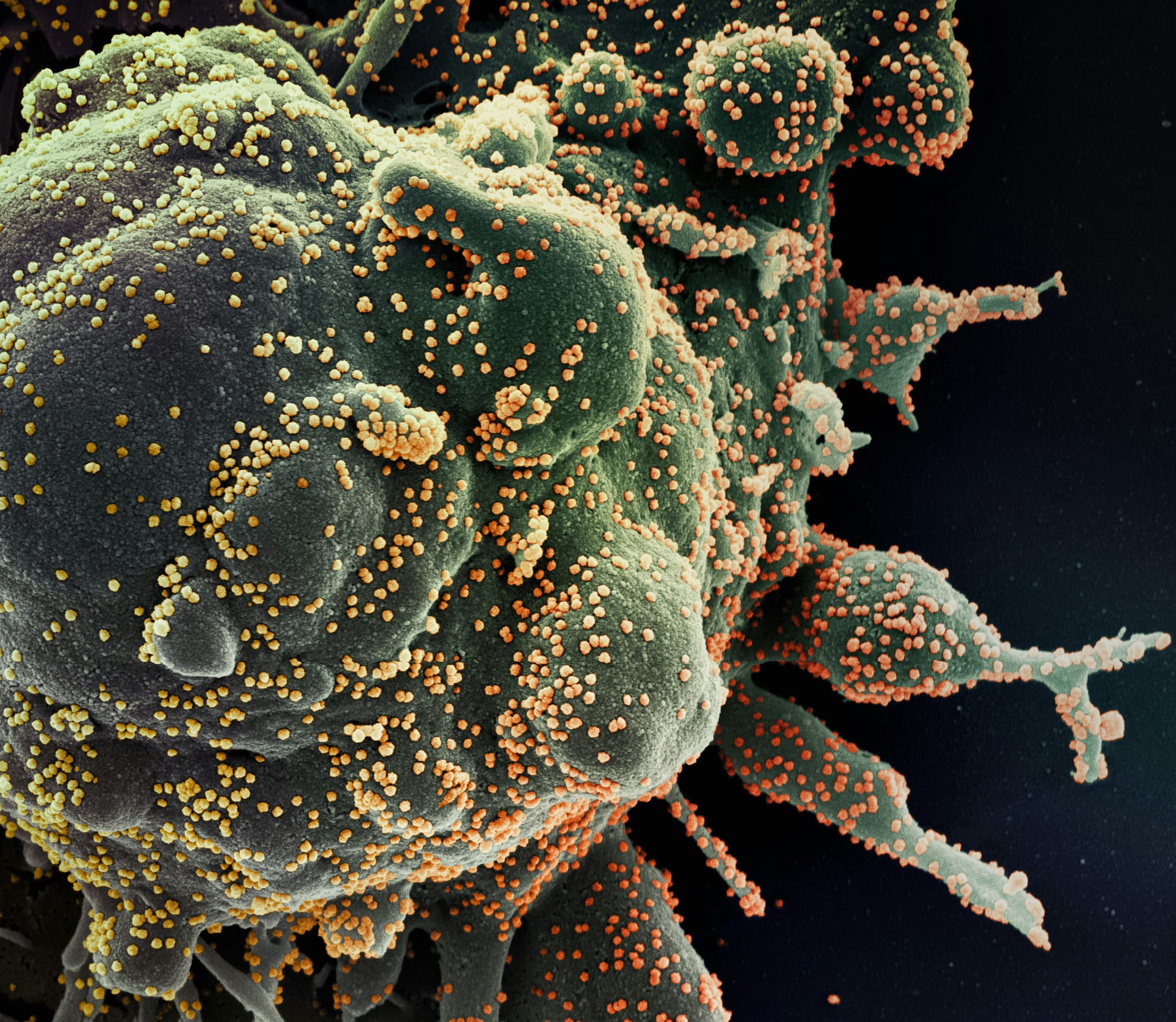
Conclusion
The transformation of commensal bacteria into pathogens is influenced by a complex range of causes, particularly host susceptibility, genetic adaptation, and environmental pressures.
Through horizontal gene transfer, bacteria gain virulence factors that enhance their ability to invade tissues, evade immune responses, and exploit intracellular niches for survival. Opportunistic infections are particularly common in immunocompromised individuals, where their weakened defences allow commensals to grow unchecked by the immune system.
Additionally, the modern increase in wide-range antibiotic treatment has accelerated the emergence of resistant strains, further complicating treatment.
Understanding these mechanisms is vital for developing solutions and prevention methods to limit the damage that these pathogens can do to the human race, ultimately protecting us for future generations.
Thank you for reading my essay on the mechanisms behind bacterial pathogenicity, bringing together examples of groundbreaking discoveries in this fascinating area of ongoing research
I hope you have thoroughly enjoyed it.
Bibliography-
Aktories, K., Schwan, C., & Jank, T. (2017). Clostridium difficile toxin biology. Annual Review of Microbiology, 71(1), 281–307. Available at: https://doi.org/10.1146/annurev-micro-090816-093458
Arias, C.A. and Murray, B.E. (2012). The rise of the Enterococcus: beyond vancomycin resistance. Nature reviews. Microbiology, 10(4), pp.266–78. Available at: https://doi.org/10.1038/nrmicro2761.
Baron, S. (2010). Medical Microbiology. [online] Nih.gov. Available at: https://www.ncbi.nlm.nih.gov/books/NBK7627/.
Belkaid, Y., & Hand, T. W. (2014). Role of the microbiota in immunity and inflammation. Cell, 157(1), 121–141. Available at: https://doi.org/10.1016/j.cell.2014.03.011
Blair, J.M., Richmond, G.E. and Piddock, L.J. (2014). Multidrug efflux pumps in Gram-negative bacteria and their role in antibiotic resistance. Future Microbiology, [online] 9(10), pp.1165–1177. Available at: https://doi.org/10.2217/fmb.14.66.
Bush, K. and Bradford, P.A. (2016). β-Lactams and β-Lactamase Inhibitors: An Overview. Cold Spring Harbor Perspectives in Medicine, [online] 6(8), p.a025247. Available at: https://doi.org/10.1101/cshperspect.a025247.
Casadevall, A. and Pirofski, L. (1999). Host-Pathogen Interactions: Redefining the Basic Concepts of Virulence and Pathogenicity. Infection and Immunity, 67(8), pp.3703–3713. Available at: https://doi.org/10.1128/iai.67.8.3703-3713.1999.
Eslami, M., Shafiei, M., Gharibi, D., Fassihian, A., & Valizadeh, S. (2021). Shiga toxin-producing Escherichia coli (STEC): A review for prevalence, pathogenicity mechanisms, surveillance, and laboratory detection methods. Microbial Pathogenesis, 150, 104675. Available at: https://doi.org/10.1016/j.micpath.2020.104675
Explorable.com. (2000). Discovery Of Bacteria - by Antony van Leeuwenhoek. Available at: https://explorable.com/discovery-of-bacteria
Falk, P. G., Hooper, L. V., & Gordon, J. I. (1998). The biology of host–microbial relationships: mapping the new frontier. Cell, 92(5), 587–590. Available at: https://doi.org/10.1016/s0092-8674(00)80949-3
Finlay, B.B. and Falkow, S. (1997). Common themes in microbial pathogenicity revisited. Microbiology and Molecular Biology Reviews, 61(2), pp.136–169. Available at: https://doi.org/10.1128/mmbr.61.2.136-169.1997.
Flynn, J.L. and Chan, J. (2001). Immunology of tuberculosis. Annual Review of Immunology, 19(1), pp.93–129. Available at: https://doi.org/10.1146/annurev.immunol.19.1.93.
Hacker, J. and Kaper, J.B. (2000). Pathogenicity Islands and the Evolution of Microbes. Annual Review of Microbiology, 54(1), pp.641–679. Available at: https://doi.org/10.1146/annurev.micro.54.1.641
Holden, V. I., Breen, P., Houle, S., Dozois, C. M., & Bachman, M. A. (2016). Klebsiella pneumoniae siderophores induce inflammation, bacterial dissemination, and HIF-1α stabilization during pneumonia. mBio, 7(5). Available at: https://doi.org/10.1128/mbio.01397-1
Lab, G. (2025). Type 3 Secretion Mechanisms and Function – Goldberg Lab. Harvard.edu. Available at: https://goldberglab.med.harvard.edu/the-lab/research/type-3-secretion-mechanisms-and-function/
Lane, N. (2015). The unseen world: reflections on Leeuwenhoek (1677) ‘Concerning little animals.’ Philosophical Transactions of the Royal Society B: Biological Sciences, 370(1666), 20140344. Available at: https://doi.org/10.1098/rstb.2014.0344
Martínez, J.L., Coque, T.M. and Baquero, F. (2014). What is a resistance gene? Ranking risk in resistomes. Nature Reviews Microbiology, 13(2), pp.116–123. Available at: doi:https://doi.org/10.1038/nrmicro3399.
Nguyen, T. L. A., Vieira-Silva, S., Liston, A., & Raes, J. (2015). How informative is the mouse for human gut microbiota research? Disease Models & Mechanisms, 8(1), 1–16. Available at: https://doi.org/10.1242/dmm.017400
Novick, R. P. (2003). Mobile genetic elements and bacterial toxinoses: The superantigen-encoding pathogenicity islands of Staphylococcus aureus. Plasmid, 49(2), 93–105. Available at: https://doi.org/10.1016/S0147-619X(02)00156-1
Paczosa, M.K. and Mecsas, J. (2016). Klebsiella pneumoniae: Going on the Offense with a Strong Defense. Microbiology and Molecular Biology Reviews, 80(3), pp.629–661. Available at: :https://doi.org/10.1128/mmbr.00078-15.
Powers, T.R., Haeberle, A.L., Predeus, A.V., Hammarlöf, D.L., Cundiff, J.A., Saldaña-Ahuactzi, Z., Hokamp, K., Hinton, J.C.D. and Knodler, L.A. (2021). Intracellular niche-specific profiling reveals transcriptional adaptations required for the cytosolic lifestyle of Salmonella enterica. PLOS Pathogens, 17(8), p.e1009280. Available at: https://doi.org/10.1371/journal.ppat.1009280.
Québatte, M., & Dehio, C. (2019). Bartonella gene transfer agent: Evolution, function, and proposed role in host adaptation. Cellular Microbiology, 21(11). Available at: https://doi.org/10.1111/cmi.13068
Sato, H., Okinaga, K. and Saito, H. (1988). Role of Pili in the Pathogenesis ofPseudomonas aeruginosaBurn Infection. Microbiology and Immunology, 32(2), pp.131–139. Available at:- https://doi.org/10.1111/j.1348-0421.1988.tb01372.x.
Stock image of a pathogen: Lee, D. (2022). What is a Pathogen? Online Learning College. Available at: https://online-learning-college.com/knowledge-hub/gcses/gcse-biology-help/pathogen/
Theriot, C.M. and Young, V.B. (2015). Interactions Between the Gastrointestinal Microbiome and Clostridium difficile. Annual Review of Microbiology, 69(1), pp.445–461. Available at: https://doi.org/10.1146/annurev-micro-091014-104115.
Vázquez-Torres, A. and Balish, E. (1997). Macrophages in resistance to candidiasis. Microbiology and Molecular Biology Reviews, 61(2), pp.170–192. Available at: https://doi.org/10.1128/mmbr.61.2.170-192.1997.
Waldor, M. K., & Mekalanos, J. J. (1996). Lysogenic conversion by a filamentous phage encoding cholera toxin. Science, 272(5270), 1910–1914. Available at: https://doi.org/10.1126/science.272.5270.1910
Wu, Z., Shao, J., Zheng, J., Liu, B., & Shen, N. (2022). A zero-sum game or an interactive frame? Iron competition between bacteria and humans in an infection war. Chinese Medical Journal, Publish Ahead of Print(16). Available at: https://doi.org/10.1097/CM9.0000000000002233